Industry Sector
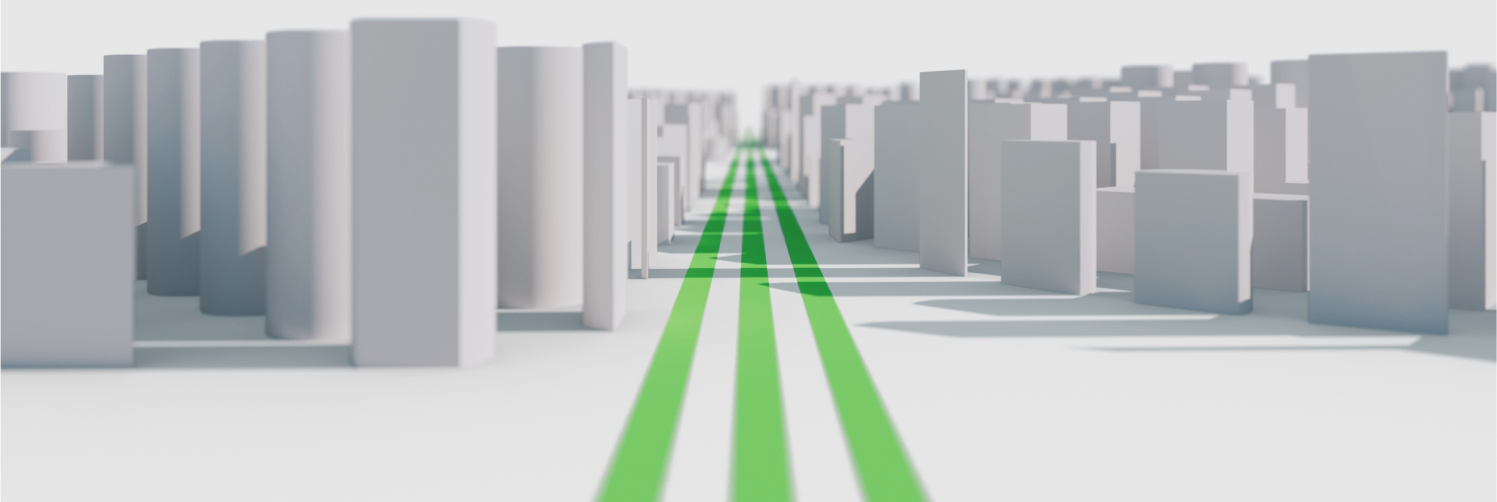
Industrial Sector Transformation in TIMES-Be
The industrial sector in TIMES-Be has been significantly enhanced and thoroughly reviewed in collaboration with industry federations and companies. As a result, the model features detailed sectoral representations, allowing for the analysis of multiple pathways to reach net-zero emissions by 2050. Key decarbonization strategies include process electrification, the adoption of low-carbon fuels and clean molecules, and the implementation of carbon capture technologies. Assuming stable industrial activity levels (e.g., consistent material production volumes), the model treats energy consumption as an endogenous variable. This methodology effectively captures interactions and synergies between the industrial sector and other parts of the energy system—such as electricity generation—supporting a cost-optimized transition for the entire energy system.
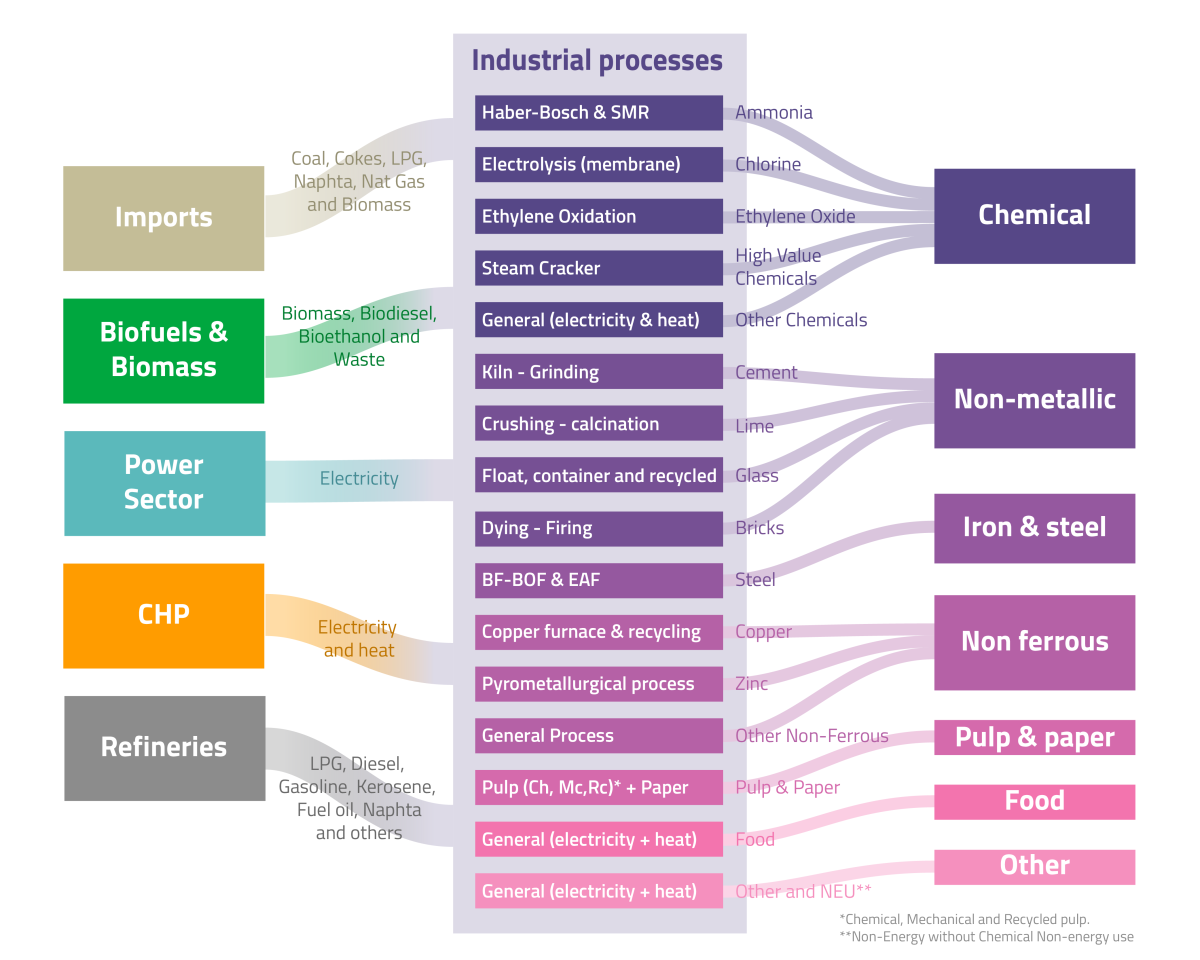
Final Energy Use
In contrast to the transport and residential sectors—where electric vehicles and heat pumps drive major reductions in final energy demand—industrial electrification does not lead to substantial demand reductions in most cases. On the contrary, more energy may be needed to decarbonize existing production processes. Nevertheless, the industrial energy mix undergoes a profound transformation, but is only 3% lower than todays levels (around 124 TWh).
By 2030, fossil fuels still make up as much as 66% of the industrial energy mix. This share declines to 50% by 2040 and further to 25–40% by 2050. Meanwhile, electricity emerges as the dominant energy carrier, with demand increasing by 50–80%—equivalent to an additional 20–30 TWh by 2050. By then, industry will account for 25–40% of Belgium’s total electricity consumption growth.
The shift toward industrial electrification unfolds in two distinct waves:
2025–2030: Early electrification of low-temperature heat via industrial heat pumps begins. Simultaneously, primary steel production shifts to Electric Arc Furnaces, based on Direct Reduction of Iron (DRI).
2045–2050: A second wave brings large-scale electrification, including electric naphtha cracker furnaces, full-electric carbon capture technologies, and electrified casting and rolling furnaces, particularly in the ROTORS scenario.
Hydrogen demand experiences a dramatic shift. By 2030, it drops from 6 TWh to 3 TWh as imported ammonia replaces half of Belgium’s local production. By 2050, its role diverges across scenarios:
In the IMPORTS scenario, hydrogen demand nearly vanishes, with full reliance on imported ammonia and no new hydrogen uses in industry.
In the ROTORS and REACTORS scenarios, hydrogen use shifts from chemicals to steel, non-ferrous metals, and non-metallic minerals, reaching 7 TWh.
In REACTORS, local ammonia production continues, requiring an additional 3 TWh of hydrogen.
For more detail on the supply and demand of molecules in the Belgian energy system, see the dedicated Molecules page.
KEY TAKEAWAYS
-
By 2040,
30-50%
Increase of final electricity demand compared to today’s levels
-
By 2040, fossil energy demand is
-30%
lower compared to 2025 (average across scenarios). A reduction of approximately 23 TWh
-
In 2050 fossil energy (natural gas) amounts to
25-40%
of total final energy consumption (33-50 TWh). Compared to 66% today (83 TWh)
-
Biomass use reaches
9-15 TWh
by 2050 (40% of total available), increasing from 3 TWh today
-
In 2050, industrial heat-pumps thermal capacity reaches
1.2 GWthor nearly 0.6 GWe
Feedstock remains fossil based
Without targeted policies promoting or economically incentivizing the use of climate-neutral carbon sources—such as waste-derived, biomass-based, or atmospheric carbon—fossil feedstock is likely to remain dominant in industrial processes, as alternatives remain more costly under the current regulatory framework.
Given the expectation of relatively stable industrial activity, total feedstock consumption is projected to remain largely unchanged. One notable exception is the partial import of ammonia (6 TWh) in ROTORS and IMPORTS, which by 2050 begins to replace natural gas as a feedstock in steam methane reforming, leading to a modest reduction in fossil fuel use. In the ROTORS scenario, methanol synthesis gains importance due to limited availability of carbon capture and storage (CCS), further reducing fossil feedstock demand for high-value chemicals.
KEY TAKEAWAYS
-
In all scenarios, fossil feedstock remains at
90 TWh
by 2050, partially replaced by clean molecules -
In ROTORS, e-methanol feedstock reaches
9 TWh
reducing fossil feedstock use by 14 TWh
Final Energy Consumption
CO2 emissions
Industrial emissions: pathway to deep decarbonization
Today, industrial CO₂ emissions in Belgium stand at approximately 27 MtCO₂, reflecting a 35% reduction compared to 1990 levels (41 MtCO₂). By 2030, all scenarios project a further reduction of around 4 MtCO₂, achieving a 46% reduction from 1990 levels. Reaching this milestone will require the industry to reduce emissions by an average of 3.5% per year over the next five years.
By 2030, significant emission reductions (over 90%) are feasible in sectors such as paper, food, and ammonia, through heat pump deployment, carbon capture, and increased imports of semi-finished products. However, emissions from high-value chemicals, non-metallic minerals, and steel are expected to remain relatively stable.
From 2030 to 2040, the pace of decarbonization diverges across scenarios:
In the ROTORS scenario, emissions decline steadily at 4% annually and reach 14 MtCO2, though limited carbon capture and storage (CCS) capacity hinders deeper reductions.
In the REACTORS and IMPORTS scenarios, average annual reductions accelerate to 14% and reach 5 MtCO2, driven by faster access to large-scale CCS and a stronger decarbonization push.
By 2050, all scenarios achieve a 95% reduction in industrial emissions relative to today. However, the pathways to net-zero diverge:
In ROTORS, a sharp 23% annual reduction is required from 2040 to 2050 to compensate for earlier CCS limitations.
In REACTORS and IMPORTS, broad CCS deployment across the chemicals, steel, and non-metallic minerals sectors accelerates the transition.
Overall, carbon capture is the dominant decarbonization strategy, contributing 50–70% of total emission reductions (13–18 MtCO₂) by 2050. The remaining 7–14 MtCO₂ of reductions are achieved through a combination of industrial electrification, biomass use, and clean molecule integration.
KEY TAKEAWAYS
-
By 2030,
4 MtCO2
emissions are reduced mainly in food, ammonia and other small industries
-
In 2040 industry will reduce emissions by
45-80%
with reference to today’s levels
-
By 2050, in all scenarios, industry emissions are
95%
lower than today and 97% with respect to 1990
-
Final energy consumption CO2 intensity in 2050 is
8-13 gCO2/kWh
compared with nearly 215 gCO2/kWh as today
The role of carbon capture in industry
Carbon capture plays a pivotal role in all three major decarbonization scenarios, with captured volumes ranging from 14 to 20 MtCO₂ annually by 2050. However, its adoption differs across sectors, influenced by factors such as CO₂ concentration in emissions, carbon pricing, and energy costs. Due to its high expense, carbon capture will be first deployed in sectors with highly concentrated emissions, such as ammonia and ethylene oxide production—with the Kairos@C project expected to drive early adoption around 2030.
Between 2035 and 2040, as the modelled CO₂ price climbs to 295 €/t, the non-metallic minerals sector is expected to significantly scale up carbon capture efforts. After 2040, adoption will expand further to include steel, high-value chemicals, and refineries.
In all scenarios, carbon storage costs outside Belgium are projected to decrease to 37 €/t by 2050, making carbon utilization less economically attractive. An exception exists in the ROTORS scenario, where carbon storage is limited to 10 MtCO₂ per year. In this case, captured fossil carbon is repurposed for methanol synthesis, embedding CO₂ into final products. It's important to mention that current EU legislation has limited mechanisms for rewarding CCU unless the CO₂ is permanently removed or embedded in long-lived products.
Additionally, small volumes of biogenic CO₂, captured from sources such as cement kilns, will be used in the production of synthetic biofuels.
By 2050, carbon capture, utilization, and storage (CCUS) could require 7–10 TWh of energy, accounting for 7–12% of total energy consumption in the sectors where it is implemented.
KEY TAKEAWAYS
-
By 2030, Carbon Capture and Storage could reduce CO2 emissions by
2 Mton/a
in the chemical sector
-
By 2040, Carbon Capture and Storage needs scaling up to
20 Mton/aIn the steel, chemical, cement, lime and glass sectors
-
Carbon Capture and Storage remains at
20 Mton/aby 2050 when not constraint
-
In Rotors, access to affordable electricity and a cap on CO2 storage leads to
9 TWh
of e-methanol production for methanol-to-high-value-chemicals processes
Industry deep dive
Steel sector
Despite ongoing industrial transformations, total final energy consumption in the steel sector remains stable, primarily due to the high energy demand of carbon capture technologies deployed by 2050. Deep decarbonization occurs mostly after 2040, driven by the adoption of carbon capture and storage (CCS) and increased electrification. The sector is analyzed in three segments: primary steel, secondary steel, and post-processing.
Primary Steel
A major shift in primary steel production begins as soon as by 2030, with a transition that leads toward 2050 to alternative production routes: 55% Direct Reduced Iron (DRI) and 45% Blast Furnace using bio-charcoal combined with carbon capture and utilization (CCU) for bioethanol production.
The DRI process uses natural gas from 2030 onwards, reducing the use of anthracite coal. In REACTORS and IMPORTS natural gas remains to be used for the DRI production route until 2050, where in ROTORS this will be replaced partially by hydrogen (up to almost 3 TWh), driven by the lower carbon storage availability and having access to lower cost hydrogen in this scenario. Carbon capture and storage is essential, combined with the DRI to reduce the emissions.
Electricity demand doubles to almost triples by 2050. Biomass demand rises to 4 TWh, used for charcoal and bioethanol.
Although switching from Blast Furnace to DRI improves energy efficiency, the high energy consumption of carbon capture partially offsets these gains. Nonetheless, the transition achieves an early 30% reduction in CO₂ intensity by 2030, lowering emissions from 1.7 tCO₂/tsteel today to 1.2 tCO₂/tsteel. By 2050, further electrification and CCS enable a 95% reduction in CO₂ intensity.
Secondary Steel
In secondary steelmaking or scrap-based production, currently 50% of energy consumption is electricity. This share remains stable until 2040, but could increase to 80% by 2050 under the ROTORS scenario. In contrast, REACTORS and IMPORTS scenarios deploy carbon capture to meet net-zero targets, achieving up to 90% emissions reduction. The enhanced electrification in ROTORS offers an alternative pathway to decarbonization, delivering a 55% reduction in emissions.
Post-Processing
Finishing and post-processing stages see limited change. Their energy consumption remains largely constant, given the distributed nature of these operations. However, under certain conditions (ROTORS), hydrogen demand could rise to 2.5 TWh, particularly for high-temperature heat applications.
Toward 2050
By 2050, deep decarbonization of the steel sector is achieved through a strategic mix of electrification, natural gas based DRI with CCS, bio-based alternatives with CCU, and clean hydrogen. The pace and technology mix differ by scenario, with some steel subsectors leaning more on CCS and others on aggressive electrification.
Chemical sector
The chemical sector is a cornerstone of industrial production, encompassing critical compounds such as ammonia, chlorine, ethylene, polypropylene, BTX, C4, and ethylene oxide. Other downstream chemical products are modeled using a streamlined, energy-intensity-based approach to reflect the diversity of their production processes.
By 2050, total feedstock demand remains stable at approximately 100 TWh, and its composition shifts only slightly. Fossil feedstock use declines but remains dominant, driven by increased ammonia imports and hydrogen-based production. Methanol emerges as an alternative feedstock for high-value chemical processes in ROTORS.
Final energy consumption increases by 6–23%, reaching 34–40 TWh by 2050 (up from 32 TWh today), largely due to the energy-intensive nature of carbon capture technologies. However, the energy mix undergoes a substantial transformation: the share of fossil energy drops to 15–30% (down from 50% today), while electricity and renewable sources become dominant, particularly after 2040, when electricity demand rises by 5–13 TWh.
Ammonia
From 2030, ammonia production shifts to a hybrid model of imports and domestic production. Until 2040, domestic production is based on blue hydrogen. After that:
In ROTORS and IMPORTS, ammonia is fully imported by 2050.
In REACTORS, domestic production continues, relying on centrally produced low-carbon hydrogen.
High-Value Chemicals
In ROTORS, naphtha crackers are electrified, requiring an additional 9 TWh of electricity. This raises final energy consumption but allows the methane by-product—traditionally used for the cracker furnace heating—to be redirected to other sectors, displacing up to 9 TWh of imported natural gas. Alternative pathways, including Methanol-to-Olefins (MTO) and Methanol-to-Aromatics (MTA), appear cost effectively in ROTORS, partially replacing conventional high-value chemical production from naphtha and diversifying the sector’s technological landscape.
In contrast, REACTORS and IMPORTS scenarios prioritize carbon capture as the primary decarbonization route. Facilities such as ethylene oxide and propane dehydrogenation plants also integrate carbon capture to further reduce emissions.
Toward 2050
Between 2025 and 2030, the sector can reduce CO₂ emissions by 24%, primarily through partial ammonia imports and carbon capture in ammonia and ethylene oxide production. By 2050, decarbonization reaches 96%, driven by a combination of:
Full ammonia import (in most scenarios),
Widespread carbon capture deployment, and
A transition in high-temperature heat sources, with growing reliance on electrification, clean molecules (in REACTORS), and bioenergy.
Steel sector takeaways
KEY TAKEAWAYS
-
Primary steel CO2 intensity changes by
-30%By 2030 and -95% by 2050 from 1,7 tCO2/t today
-
Secondary and post-processing steel emission are
25-70%
lower by 2050
-
Steel production will require
4-7 TWhmore electricity by 2050 than in 2025. An increase of 100-160%
-
Steel will consume
4 TWhof biomass in 2050.
This is 10-20% of the assumed total available biomass in Belgium
-
By 2050, fossil energy covers
40-60%
of total energy consumption. Around 55% in primary steel, and 20-60% in secondary and post-processing
Chemical sector takeaways
KEY TAKEAWAYS
-
Emissions are reduced
96%by 2050. By 2040, reduction ranges from 35-70%
-
By 2050, electricity consumption increases
5-13 TWh
In particular when naphtha furnaces are electrified (9 TWh)
-
Fossil energy demand is reduced by
40-65%
in 2050 compared to today's levels
-
Solid biomass consumption reaches
5 TWh
in 2050 for energy purposes, 15% of the total available biomass